Slot Thailand
IDXSTAR ✅ Situs Slot Server Thailand Super Gacor Gampang Maxwin No 1
IDXSTAR ✅ Situs Slot Server Thailand Super Gacor Gampang Maxwin No 1
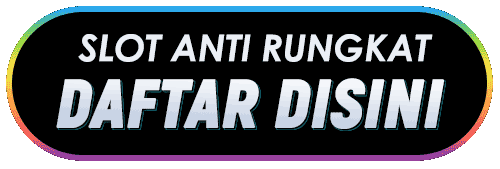
IDXSTAR adalah salah satu situs slot server Thailand terpercaya di Indonesia saat ini yang memiliki beraneka ragam jenis permainan slot online gacor paling populer. Bermain di situs slot Thailand IDXSTAR, pasti pemain akan merasakan banyak sensasi permainan yang seru dan menantang. Karena IDXSTAR merupakan penyedia permainan slot gacor gampang maxwin dan juga menyediakan akun pro Thailand terbaru menjelang tahun 2024 yang akan memberikan banyak sekali keuntungan.
Bagi pemain slot server Thailand yang memang mencari server luar negeri terbaik, di IDXSTAR merupakan pilihan tepat yang tidak mungkin membuat Anda kecewa. Sebagai penyedia situs slot Thailand IDXSTAR terbaik, IDXSTAR sangat mudah diakses oleh kalian para pemain slot88 resmi gacor yang ada di Indonesia. Maka dari itu sudah jelas sekali jika slot server Thailand IDXSTAR merupakan solusi paling jitu yang bisa Anda mainkan untuk meraih kemenangan yang besar.
Informasi Slot Thailand Gacor Hari Ini Profit Asli Terbesar
Untuk para penikmat permainan slot online akun pro Thailand di Indonesia tentu saja memerlukan informasi seputar permainan apa saja yang sedang gacor hari ini dan bisa dimainkan di waktu yang tepat. Jadwal slot gacor yang akan diberikan merupakan jadwal yang sudah fix di seputaran jam berikut di bawah ini.
- Gate of Olympus : Jam Gacor Slot Server Thailand 22.00 - 00.00 | 03.30 - 05.45 | 08.30 - 10.30
Permainan Gate of Olympus adalah salah satu produk pragmatic play yang menjadi maskot terbaik yang sering kali memberikan bonus kemenangan terbesar x500 maxwin slot Thailand.
- Sweet Bonanza : Jam Gacor Slot Server Thailand 16.30 - 19.00 | 22.30 - 01.00 | 04.30 - 06.30
Sweet Bonanza juga merupakan game slot gacor besutan pragmatic play yang sangat ikonik sekali memberikan nuansa permainan yang begitu seru dan memiliki fitur beli freespin yang sangat rendah.
- Mahjong Ways 2 : Jam Gacor Slot Server Thailand 14.00 - 20.00 | 21.00 - 00.00 | 03.00 - 05.00
Mahjong Ways 2 merupakan game slot online keluaran produk PG Soft yang sangat ini booming sekali disukai para pecinta permainan slot online server Thailand.
Beberapa game slot server Thailand yang sudah disebutkan di atas bisa menjadi acuan kalian dalam bermain game slot online dan juga bisa dilihat langsung dari situs rtp IDXSTAR akun pro Thailand yang diupdate setiap hari secara otomatis mengikuti pola mesin setiap provider game slot online yang ditawarkan secara live sehingga setiap pemain memiliki kesempatan yang sama dalam memperoleh kemenangan.
Kelebihan Bermain Slot Thailand Gacor Di IDXSTAR
Sebagai penyedia game slot online server Thailand terbaik di Indonesia, IDXSTAR menawarkan berbagai jenis game slot dari berbagai jenis provider terbaik di Asia yang sudah memiliki popularitas dan predikat yang sangat baik sehingga dapat dipercaya oleh semua penikmat game online. Beberapa kelebihan yang bisa didapat ketika Anda bermain di situs slot Thailand IDXSTAR yaitu:
- Kami memberikan pelayanan responsif selama 24 jam penuh bahkan Anda bisa berkonsultasi terlebih dahulu kepada tim customer service kami.
- Link akses slot gacor yang mudah tanpa VPN, Anda bisa menikmati setiap game online yang ditawarkan mulai dari judi bola, live casino, poker online, slot online, togel, tembak ikan hingga sabung ayam.
- Banyak bonus promosi untuk membaru baru dan lama.
- Tersedia fitur RTP Live Slot Gacor hari ini yang akan diupdate setiap harinya sesuai dengan provider yang tersedia.
- Jackpot besar dan mudah maxwin sudah pasti bisa Anda dapatkan di situs slot Thailand IDXSTAR menggunakan akun pro Thailand resmi.
Sebagai salah satu agen judi slot online akun pro Thailand di Indonesia yang bekerja sama dengan puluhan provider game online ternama dan terbaik di Asia. IDXSTAR berhasil membangun sebuah kepercayaan dengan pencipta game online khususnya slot server Thailand untuk membangun sebuah website judi online slot gacor server Thailand dan juga dikenal sebagai slot gacor maxwin yang sudah dipercaya oleh masyarakat Indonesia.
Pada intinya, segera lakukan pendaftaran untuk mendapatkan akun pro Thailand resmi di situs slot gacor IDXSTAR. Informasi ini diberikan dengan tujuan supaya Anda mengetahui profil dari sebuah website IDXSTAR sehingga Anda pun akan mendapatkan kenyamanan dan keamanan sebelum mulai melakukan pendaftaran dan deposit di situs slot Thailand terbaik di Indonesia.
Couldn't load pickup availability
Share
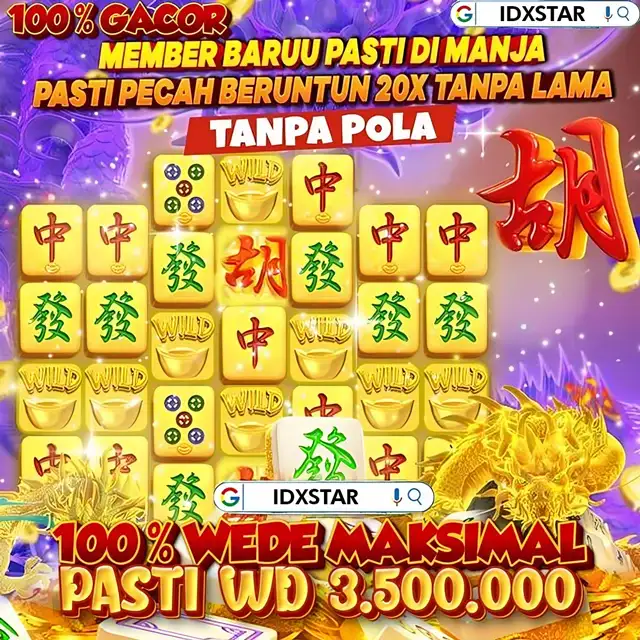